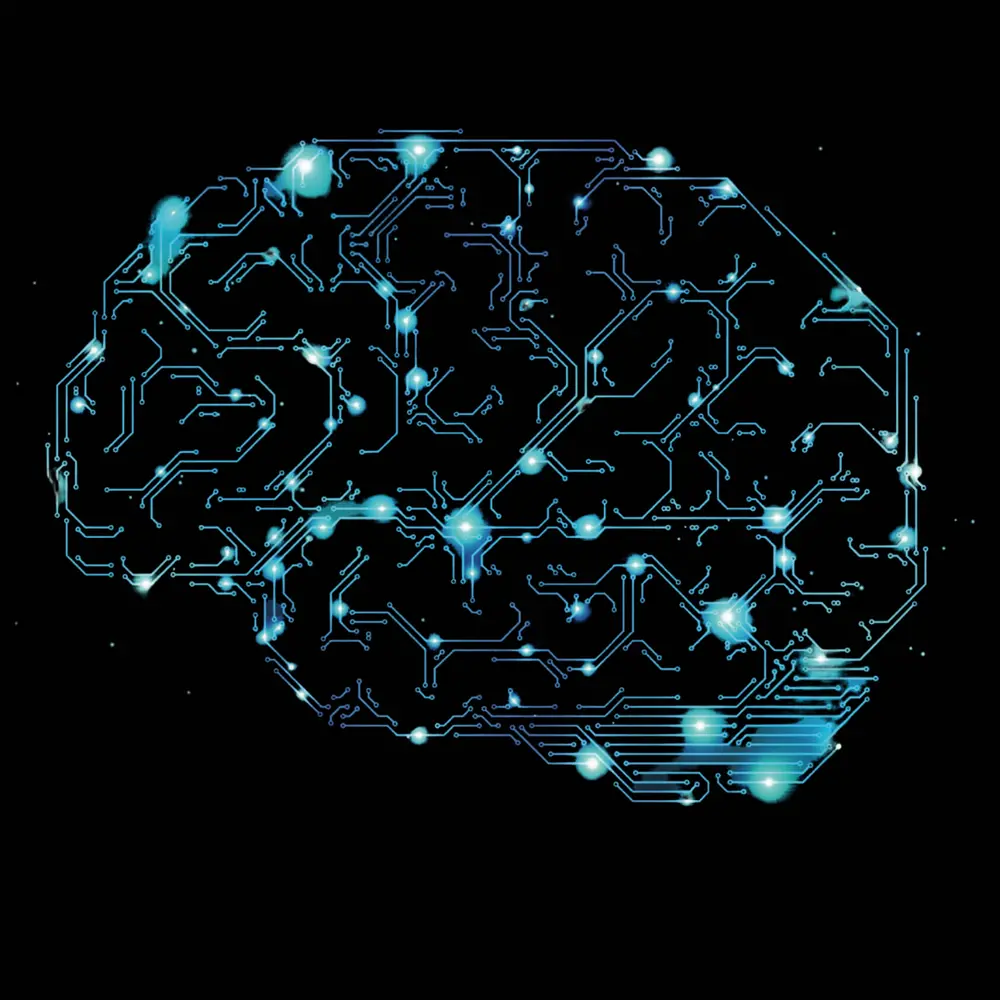
A new era has dawned in understanding neuropathological disorders such as epilepsy, schizophrenia, depression, and autism spectrum disorders. These disorders, traditionally classified in the Diagnostic and Statistical Manual of Mental Disorders by the American Psychiatric Society, are now being re-examined through the lens of molecular and cellular physiology. As a result of novel research techniques, they are increasingly being regarded as neurophysiological disorders, and the stigmatization directed towards those diagnosed with these disorders is decreasing.
It is difficult to assess the total impact of neuropathological disorders. One study, for example, found that the economic cost of depression in America is $210 billion per year and that for every dollar spent in its treatment, about $6.60 is spent on costs of related illnesses, reduced workplace productivity and suicide (Greenberg, Scientific American, 2015). Epilepsy, the most common neurologic condition after headache, is associated with a risk of death which is 2-3 times higher overall than in the general population (Wiebe, Goldman's Cecil Medicine 24th edition, 2012) and is also frequently associated with depression, reproductive dysfunction and generally poor psychological and physical outcomes. In 1990 the total economic cost of schizophrenia was estimated to be $32.5 billion and $46.6 billion for anxiety disorders. (Rice, J Clin Psychiatry, 1999) These data just brush the surface of the total impact of these disorders and of others such as autism spectrum disorders.
Altered neuronal excitability is the common denominator in this wide spectrum of neuropathological disorders, and scientists in the University of Illinois Department of Molecular and Integrative Physiology (MIP) are in the forefront of this research activity. Utilizing a multidisciplinary approach, the laboratories of Drs. Catherine A. Christian, Nien-Pei Tsai, and Hee Jung Chung are studying neurobiological phenomena that are not only important in disease but also are essential in learning and memory.
The diagram below, demonstrating the anatomy of a neuron, the cell which processes information in the central nervous system, is basic to understanding this research. The neuron has processes which receive and transmit information, the dendrites and the axons, respectively. The tiny gap between neurons through which information signals flow is known as the synapse, truly a key area in information processing. The inside of the neuron is electrically negative with respect to its outside, with a resting membrane potential of approximately -60 mV. When an action potential is generated, it depolarizes the neuronal cell membrane and is transmitted down the axon and through the synapse. The cell body of the neuron manufactures chemical neurotransmitters which are transported to the axon terminal, and these are released in the synapse as a result of the conduction of electrical signals along the axon. There are two types of synapses, those which transmit electrical signals only, and the much more common chemical synapse. When neurotransmitters are released, they bind to receptors on the postsynaptic neuron and influence the activity of ion channels, essential elements in synaptic transmission. The membrane potential of the postsynaptic neuron is changed as a result, and depending on the receptors activated by the neurotransmitters, can either raise or decrease the level of activity of the postsynaptic neuron.
Neurons are able to monitor their activity (i.e. firing rates) through calcium-dependent sensors which actually regulate the number of neurotransmitter receptor sites at synapses in addition to the amount of neurotransmitter that is released. (Turrigiano, Cold Spring Harbor Perspect Biol, 2012) To add to the complexity of this feedback system, there are additional mechanisms that the nervous system utilizes to modify firing rates not only at local synaptic sites but also globally at network-wide locations.
The capacity of self-monitoring and self-regulation of the central nervous system's activity is known as synaptic plasticity, a truly exciting concept (Whalley, Nat Rev Neurosci., 2007). The synapse, once regarded as fixed and unchangeable in recent decades, has actually been proven to be plastic, accommodating to various environmental conditions by reacting to neurotransmitters that are released to strengthen or weaken neuronal activity. Dr. Tsai is studying the role of protein translation and degradation in plasticity. Neuronal activity modulate the dynamics of proteins which are involved in neurodevelopmental events, and when this process is dysfunctional various neurodevelopmental disorders can result. Synchronous (global) firing of neurons is critical to learning and memory, but abnormalities in control of this synchrony are associated with a spectrum of disorders, including epilepsy, depression, autism spectrum disorders, and schizophrenia.
One of the challenges in studying neuropathological disorders has been the identification of specific genes and signaling pathways associated with the disorders and the articulation of gene-environment interactions. A recent paper published in the journal Molecular Brain by Dr. Tsai’s lab in collaboration with Dr. Christian, however, describes, for the first time in the literature, a molecular signaling feedback pathway critical to understanding epilepsy (Jewett et al., Mol Brain, 2016). They have pharmacologically stimulated neuronal activity and demonstrated that this triggers an enzyme (Mdm2) to degrade a transcription factor p53. p53 normally suppresses an epilepsy-associated gene Nedd4-2 to produce messenger RNA, which results in enhanced seizure susceptibility. Through in vitro and in vivo mouse studies, they have demonstrated that when p53 is inhibited, seizure susceptibility in mice is inhibited. However, Dr. Tsai’s lab has demonstrated that when the degradation of p53 is blocked neural network synchrony is enhanced. When p53 is inhibited, neural network synchrony is reduced. These data demonstrate a specific molecular regulatory feedback loop controlling neuronal excitation in the healthy state (Figure 1).
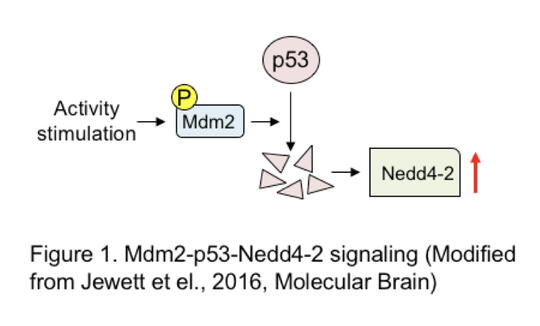
Dr. Tsai’s lab has shown the crucial importance of the Mdm2-p53-Nedd4-2 signaling pathway as a feedback loop in controlling neuronal excitation and synchrony. The evidence that both p53 and the epilepsy-associated gene Nedd4-2 are linked to seizure susceptibility affords a potential for novel therapeutic strategies. Their focus on dysregulated neuronal excitation, specifically related to Mdm2, also has potential importance in understanding and treating other conditions such as autism spectrum disorders.
An interesting facet of Dr. Christian's epilepsy research focuses on the association of female reproductive dysfunction with epilepsy. Using mouse seizure models, her laboratory was able to show an association between estrous cycle disruption and abnormally elevated neuronal activity in the hypothalamic gonadotropin-releasing hormone neurons, the central neurons that control reproduction. Further research concerning hormone production in males and females will further our understanding of the relationship between seizures and reproductive function and lead to new therapeutic targets.
A fascinating area of research centers on the role of astrocytes, a type of glial cell that have traditionally been regarded simply as cells that support neurons (Halassa et al., Trends Mol Med, 2007). Interestingly, the cellular processes of astrocytes make contact with both the pre- and the post-synaptic neurons, allowing not only assessment of vascular supply but also of neuronal activity. In recent years, the role of astrocytes in actively enhancing or reducing synaptic transmission is being recognized (the "astrocytes activation spectrum"), contributing to epilepsy and schizophrenia, respectively. This is accomplished through calcium signaling and variable levels of release of gliotransmitters such as glutamate and D-serine. Dr. Christian's laboratory is focusing on the role of astrocytes in modulating synaptic inhibition by studying GABA receptors, key inhibitors in the vertebrate central nervous system. The goal of these studies is to promote understanding of how these receptors and specific endogenous peptides (short sequences of amino acids) influence abnormal behaviors.
Dr. Hee Jung Chung's research centers on the role of ion channels, key elements in controlling neuronal excitability and synaptic communication. Dr. Chung's epilepsy research focuses on mutations in KCNQ potassium (K+) channels that cause mild-symptomatic to drug resistant epilepsy in children. These potassium channels localized at the neuronal axon are inhibitory since they keep the membrane potential more negative than the threshold potential for action potential firing. Dr. Chung's research discovered that epilepsy mutations block the channel expression at the neuronal axon as well as channel opening, leading to excessive firing of hippocampal neurons.
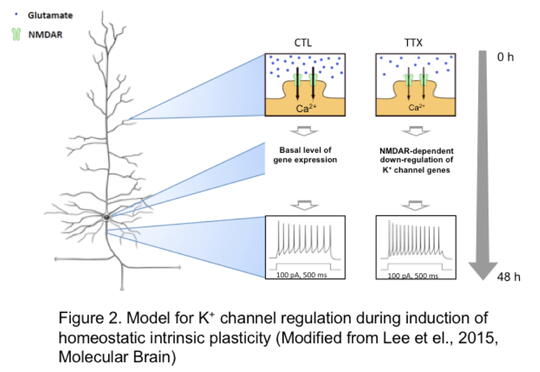
Dr. Chung's laboratory also studies the normal role of ion channels in maintaining the dynamic control of neuronal excitability and synaptic communication. Adaptation to a constantly changing environment requires plastic but also stable neuronal circuits. Dr. Chung's lab has identified a rich gene network that control such adaptation and that are regulated by NMDA-type glutamate receptors that mediate Ca2+ ions to move into the neuron (Lee et al., Mol Brain, 2015). NMDA receptor-dependent sensing of the activity level of the hippocampal neurons in turn leads to regulation of the genes that produce multiple K+ channels. The resulting current through these K+ channels modifies the rate of firing of the neurons in the network (Figure 2). The fact that mutations in specific K+ ion channels are associated with epilepsy strongly suggests that Dr. Chung's work could point to potential therapeutic targets. In addition, her laboratory's discovery of novel genes that influence synaptic strength and calcium sensors could add to the armamentarium of therapeutic agents.
The research projects highlighted in this article illuminate various mechanisms which achieve this balance through accurate sensing of network activity (e.g. Ca2+-dependent sensors), controlling the types and amounts of proteins produced by specific genes, and controlling ion channel activities. The type and quality of research studying neuronal excitability in health and disease that is underway in our department perfectly embodies our acronym (MIP)...it is truly molecular and integrative physiology.